The Remarkable Properties of Neutron Stars
Note: An earlier version of this article appeared on this blog by Peter Edmonds.
The collapse of a massive star in a supernova explosion is an epic event. In less than a second a neutron star (or in some cases a black hole) is formed and the implosion is reversed, releasing prodigious amounts of light that can outshine billions of Suns. That is a spectacular way to be born. Here, I'll explain that the properties of neutron stars are no less spectacular, even though they are not as famous as their collapsed cousins, black holes.
Because of the incredible pressures involved in core collapse, the density of neutron stars is astounding: all of humanity could be squashed down to a sugar cube-sized piece of neutron star. The escape velocity from their surface is over half the speed of light but an approaching rocket ship would be stretched, then crushed and assimilated into the surface of the star in a moment. Resistance would be futile.
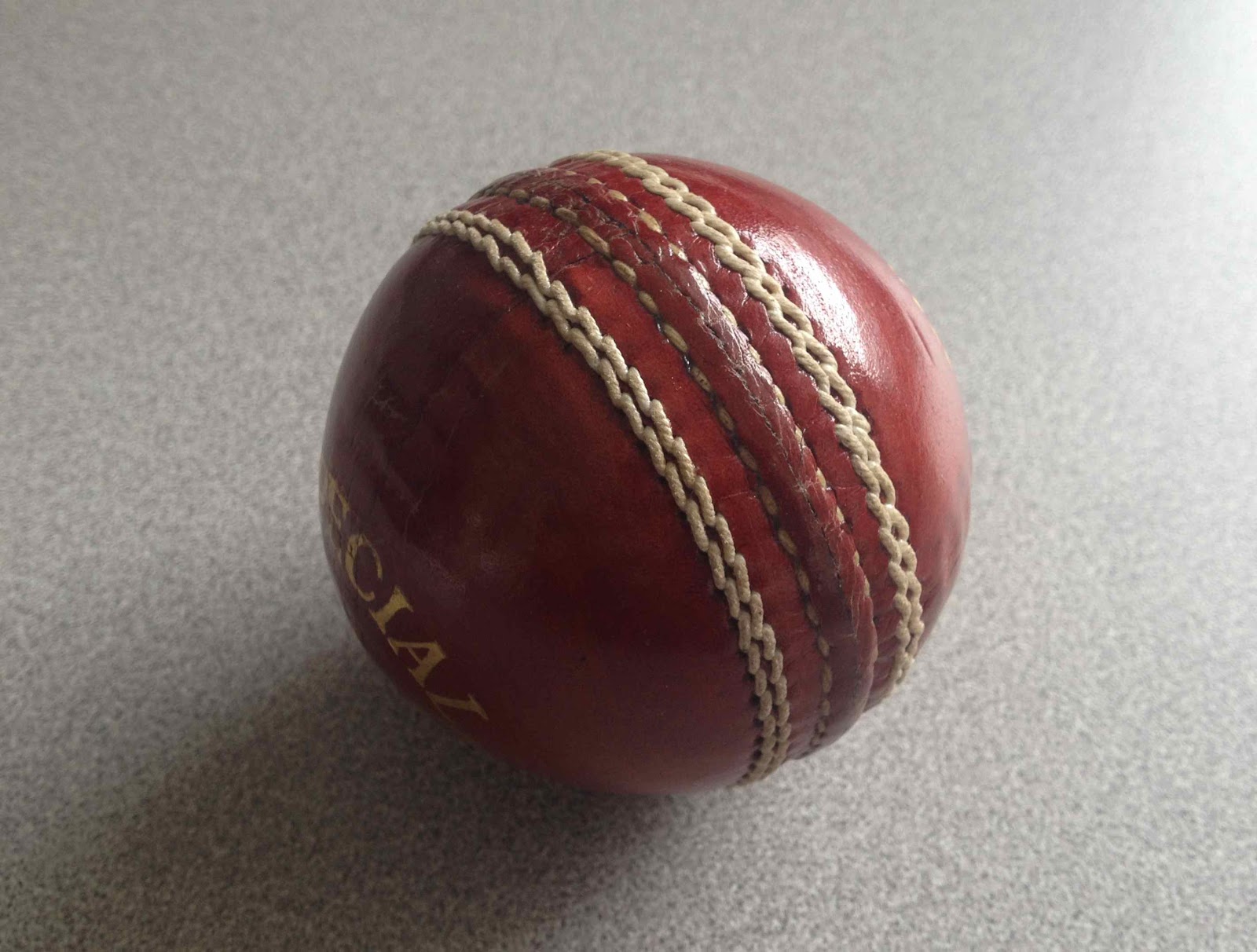
If this cricket ball were made of neutron star material it would weigh about 20 trillion kg, or about 40 times the estimated weight of the entire human population.
Another remarkable property is that neutron stars generate the most extreme magnetic fields known in the universe, up to a quadrillion times the strength of Earth's magnetic field. If one of these ultra-magnetic neutron stars, called a magnetar, flew past Earth within 100,000 miles, its magnetic field would destroy the data on every credit card on Earth. Luckily for our economy none are that close, but the distant ones can put on spectacular shows. In 2004 a magnetar underwent an extraordinary outburst and become one of the brightest objects ever observed in the sky, causing a disturbance in the Earth's ionosphere that was recorded around the globe, as described in this paper by astrophysicist Bryan Gaensler (@SciBry on Twitter) from the University of Sydney (my alma mater). That's impressive for an object about the size of a city that is located around 50 thousand light years away.
An artist's conception of the spectacular outburst from the magnetar SGR 1806-20, including magnetic field lines. After the initial flash, smaller pulsations in the data suggest hot spots on the rotating magnetar’s surface. This animation contains no audio, because "in space no-one can hear you scream". Credit: NASA
Neutron star behavior can be so odd and distinctive that their discovery was initially greeted as the possible discovery of extraterrestrial intelligence. The real explanation is that a pulsar, a rotating neutron star, was discovered. Pulsars have become such an important tool for physics research that two different Nobel Prizes have been awarded in their name, the first for their discovery by Antony Hewish. Many people - including myself - have argued that Jocelyn Bell Burnell should have been awarded part of the Nobel prize with Hewish, since she made the discovery, but in an expression of modesty or Imposter Syndrome, Bell Burnell later commented that she did not deserve the award. However, this does not diminish the significance of her discovery, and of the outstanding research that it enabled.
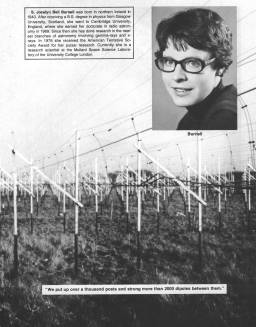
Jocelyn Bell Burnell with the radio telescope she used to discover pulsars. Credit: Jocelyn Bell Burnell.
The second Nobel prize was for Russell Hulse and Joseph Taylor, who discovered the first known binary pulsar, PSR1913+16, which has become extremely valuable for testing Einstein's Theory of General Relativity (GR). Since then other important objects have been discovered, including a double pulsar system known as PSR J0737-3039A/B that is one of the best objects available for testing GR and alternative theories of gravity, as explained in this paper by Michael Kramer from the University of Manchester.
Looking ahead in pulsar work, there is an exciting and ingenious project called the North American Nanohertz Observatory (NANOGrav) that is attempting a direct detection of gravitational waves, ripples in the fabric of space-time, using pulsars. Like several of the topics covered here this project deserves a dedicated blog post, but for now I'll just say that exotic objects like black hole binaries are expected to produce gravitational waves. Two of the pulsar experts leading this project are Scott Ransom from NRAO, whose enthusiasm for pulsars is well explained by the papers he writes, like this one: "Pulsars are cool. Seriously" and Victoria Kaspi, from McGill University, seen here speculating about some possible applications of pulsar research.
So far I've emphasized spectacular features of neutron stars and some famous results. These stories capture a lot of attention and do an excellent job at promoting astrophysics, but most research occurs in the gaps between catchy headlines and Nobel prizes. These gaps contain plenty of room for excellent research, much of it about understanding the nature of neutron stars, rather than testing fundamental physics with them.
Some of the most important open questions about neutron stars concern their size and structure. How large are they? What makes up their atmosphere? What is their core like?
One key advantage that neutron stars have over black holes is that their surface is visible to us, enabling much to be be learned about their atmospheres and interior structure. For example, in 2009, Wynn Ho from the University of Southampton and Craig Heinke from the University of Alberta, found evidence for a carbon atmosphere on the neutron star in the Cassiopeia A supernova remnant, using NASA's Chandra X-ray Observatory (note: I work at the Chandra X-ray Center in the Education and Public Outreach Group). This resolved a mystery about the nature of the neutron star, as the press release and Nature paper explain. An interesting side-note: the researchers calculate that the carbon atmosphere is only about 4 inches thick, as shown in the figure, because it has been compressed by a surface gravity that is 100 billion times stronger than on Earth. We're used to talking about massive scales and distances in astronomy, not small ones.
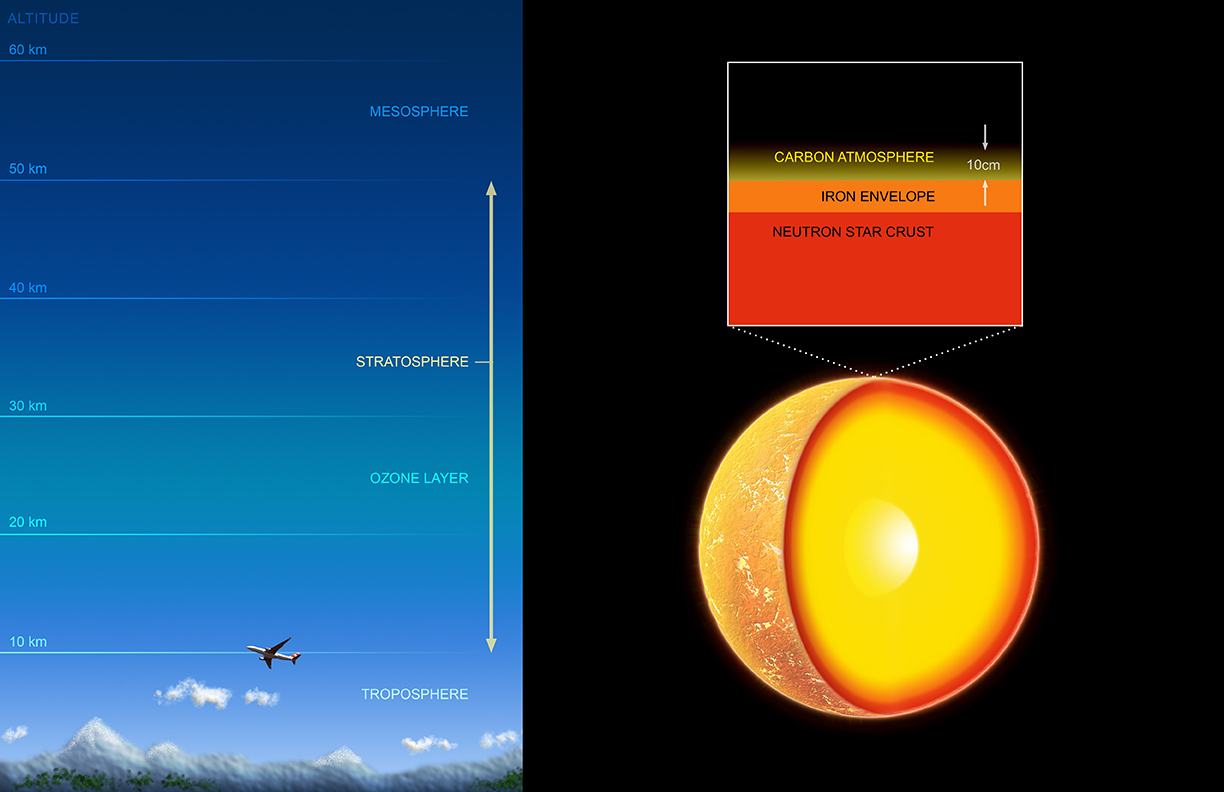
The properties of the carbon atmosphere on the neutron star in the Cassiopeia A supernova remnant are remarkable. It is only about four inches thick, has a density similar to diamond and a pressure more than ten times that found at the center of the Earth. As with the Earth's atmosphere, the extent of an atmosphere on a neutron star is proportional to the atmospheric temperature and inversely proportional to the surface gravity. The temperature is estimated to be almost two million degrees, much hotter than the Earth's atmosphere. However, the surface gravity on Cas A is 100 billion times stronger than on Earth, resulting in an incredibly thin atmosphere. Caption taken from Chandra web-site. Credit: NASA/CXC/M.Weiss
Heinke and Ho followed up this work with an even more interesting result, the first direct evidence for a superfluid, a bizarre, friction-free state of matter, at the center of a neutron star. First, a 4% drop in the temperature of the Cas A neutron star over 10 years was observed with Chandra and reported by Heinke et al. Then, two different papers, one in Physics Review Letters led by Dany Page from the National Autonomous University in Mexico and another in Monthly Notices of the Royal Astronomical Society led by Peter Shternin from the Ioffe Institute in St Petersburg, Russia independently came up with the same explanation. When the temperature of the neutron star fell below a critical level, a superfluid formed in the core of the star, forming neutrinos which travel outwards, taking energy with them. This causes the star to cool rapidly as observed with Chandra.
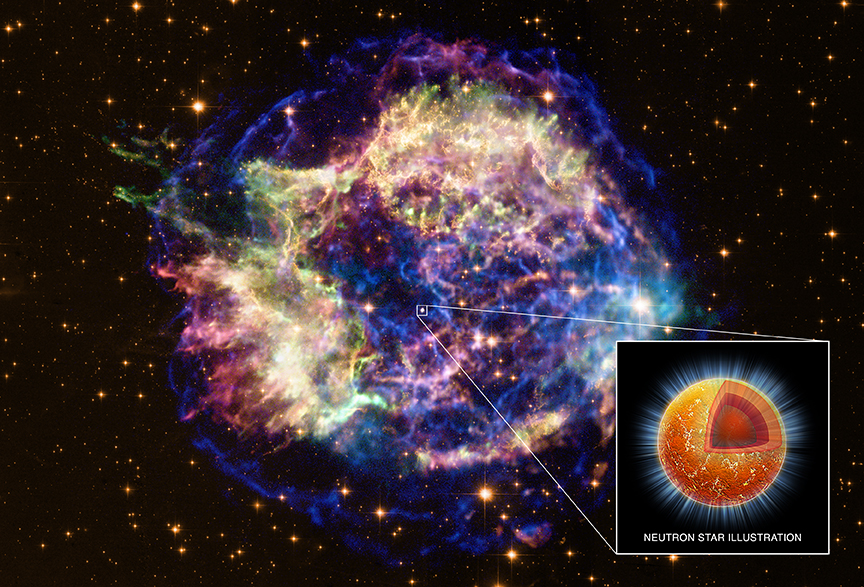
This image shows a composite of X-rays from Chandra (red, green, and blue) and optical data from the Hubble Space telescope (gold) of Cassiopeia A, the remains of a massive star that exploded in a supernova. The artist’s illustration in the inset shows a cutout of the interior of the neutron star where densities increase from the crust (orange) to the core (red) and finally to the part of the core where evidence for a superfluid has been found (inner red ball). The blue rays emanating from the center of the star represent the copious numbers of neutrinos -- nearly massless, weakly interacting particles -- that are created as the core temperature falls below a critical level and a neutron superfluid is formed, a process that began about 100 years ago as observed from Earth. These neutrinos escape from the star, taking energy with them and causing the star to cool much more rapidly. Credit: X-ray: NASA/CXC/UNAM/Ioffe/D.Page,P.Shternin et al; Optical: NASA/STScI; Illustration: NASA/CXC/M.Weiss
Other important information about the structure of neutron stars comes from studying the relationship between their size and mass. For a given mass, the size of a neutron star will depend on how stiff or soft the structure is. These are all relative terms, since by Earthly standards, nothing about neutron stars is soft.
Old neutron stars are typically faint objects, but when they pull material away from companion stars they can become much brighter, allowing good studies of their atmospheres. Observations of the amount of X-rays at different wavelengths, combined with theoretical models for their atmospheres, can allow the relationship between the radius and mass of the neutron star to be estimated. This work has been performed by Heinke, by Natalie Webb and Didier Barret (both from the Institut de Recherche en Astrophysique et Planétologie) as explained in this paper, and by Sebastien Guillot (Seb_Guillot on Twitter) from McGill University, in this paper. All of these observations were of neutron star binaries in globular clusters.
Neutron stars pulling material away from companions have also been observed to undergo bursts of X-rays, caused by thermonuclear explosions on their surfaces. These explosion can cause the atmosphere of the neutron star to expand. If observers catch one of these bursts they can follow as the star cools and calculate its surface area. When this area is combined with independent estimates of the distance to the neutron star, the relationship between the mass and radius of this object can be estimated. Two researchers who have applied this technique with great success are Feryal Ozel from the University of Arizona and Tolga Guver from Sabanci University, as described in this set of papers here, here, here, and here.
Each of the papers quoted in the previous two paragraphs provide information about the mass and radius of the neutron star and about their structure. However, there may be problems with relying too much on a single technique or a single object. A very good new paper by Andrew Steiner, from University of Washington, avoids this problem by combining all of the papers mentioned above: 4 neutron stars in globular clusters quietly pulling material from a companion and 4 undergoing X-ray bursts.
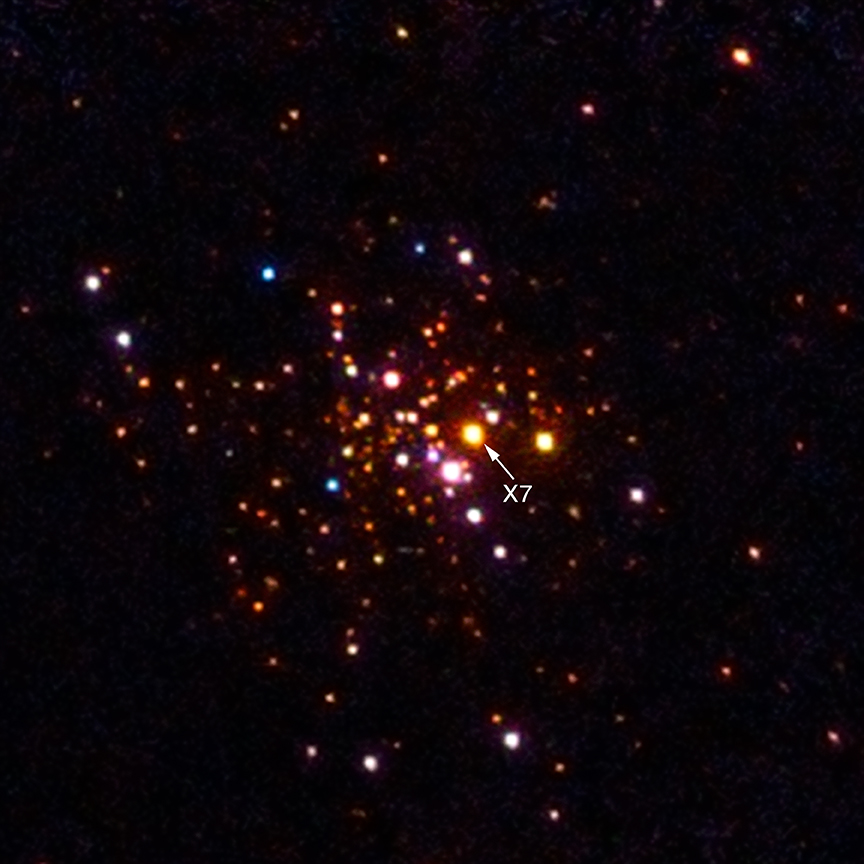
A Chandra X-ray Observatory image of 47 Tucanae, my favorite globular cluster. One of the neutron star binaries from Steiner et al. (2013), called X7, is labeled. Credit: NASA/CXC/Michigan State/A.Steiner et al.
Steiner et al. take these results and apply the latest neutron star models to estimate that the radius of a neutron star with a mass that is 1.4 times the mass of the Sun - a typical value - is between 10.4 and 12.9 km (6.5 to 8.0 miles), as we reported recently in a Chandra image release. They also estimate that the density at the center of a neutron star is almost ten times that of nuclear matter found in Earth-like conditions. This is equivalent to a pressure that is over ten trillion trillion times the pressure required for diamonds to form inside the Earth.
Using their results, Steiner et al. are able to compare their results with values derived from nuclear physics experiments performed on Earth, such as the distance between protons and neutrons in atomic nuclei. A larger neutron star radius implies that, on average, neutrons and protons in a heavy nucleus like Uranium are farther apart.
What is the core of a neutron star made of? It could be neutrons or it could be free quarks, the fundamental particles that combine to form protons and neutrons but which are not usually found in isolation. The paper by Steiner et al. cannot distinguish between these two possibilities, but there is potential to do so with future neutron star work.
There are many other interesting and important results about neutron stars. Regarding their structure, there are the very strong constraints that have come from pulsar work, such as the mass measurement of (1.97+/-0.04) solar masses by Demorest et al. (2010), with Scott Ransom as a co-author, that has already accumulated over 400 citations! An even larger neutron star mass might have been found by Romani et al. (2012). Then there are the astonishing spin rates that these incredibly massive, city-sized objects can reach, such as PSR J1748-2446ad which spins around 716 times a second, as reported by Hessels et al. (2006), with Ransom and Victoria Kaspi as co-authors.
I will continue to follow developments in neutron star research closely, as part of my job with Chandra but also because of my excellent location at Harvard-Smithsonian Center for Astrophysics (CfA), which has a regular stream of visitors covering a wide range of astrophysics. For example, Scott Ransom is giving a talk in early April about NANOGrav.
In the meantime, I may write a blog post or two on black holes, which are rumored to be interesting objects.
Please note this is a moderated blog. No pornography, spam, profanity or discriminatory remarks are allowed. No personal attacks are allowed. Users should stay on topic to keep it relevant for the readers.
Read the privacy statement